Pictures of the Day CH320M/CH328M
9-11-24
The Unique Structure of Amide Bonds
Amides have structural characteristics that are unique among carboxylic acid derivatives. Had you been asked last week to describe the geometry of an amide bond, you probably would have predicted bond angles of 120° about the carbonyl carbon and 109.5° about a tetrahedral amide nitrogen. In the late 1930s, one of Linus Pauling’s most important discoveries was that the geometry of the nitrogen atom of an amide bond in proteins is closer to trigonal planar (sp2), not tetrahedral (sp3). A large number of subsequent experiments have verified that amides should be thought of as being best represented as the resonance hybrid of three resonance contributing structures, not two like other carbonyl species. In the unique third contributing structure of an amide, the lone pair on nitrogen donates electron density to create a pi bond between carbon and nitrogen.
When you look back at your resonance contributing structures homework, you
will notice something tricky. As in the case of the enolate ion shown above,
the hybridization states for certain atoms are predicted to be different
in different contributing structures. Since hybridization state does not
really change, how do you decide which is the correct hybridization state?
The answer is that in cases of resonance contributing structures in which
a given atom is either sp3 or sp2 hybridized, the rule is that if
any significant contributing structure indicates an atom is sp2, then sp2
is the hybridization state of the atom. In the example above, this
means that the partially negatively charged carbon and oxygen atoms are both
sp2 hybridized, even though they appear sp3 in one contributing structure
each.
The reason for this "sp2 dominates" rule is that pi
electrons prefer to be delocalized, and this can only occur if all
the adjacent 2p orbitals overlap. In other words, molecules are more stable
if pi electrons are delocalized over more than jsut two atoms. There are
no 2p orbitals around an sp3 hybriduzed atom, so this stabilizing pi electron
delocalization can only take place when the atoms are sp2 hybridized and
the geometry is such that the 2p orbitals overlap. Note that with the electron
delocalization in the pi orbitals, you
also get charge delocalization, further stabilizing the overall structure.
Thus, by adopting an sp2 geometry, the enolate ion is more stable (stabilization
from both pi electron and charge delocalization), so sp2 is the hybridization
that is observed. This is why the cases of resonance contributing structures
you have seen involve the movement of electron pairs between pi bonds and
lone pairs or between adjacent pi bonds. No single Lewis structure accurately
conveys that the pi electrons are delocalized over more than two atoms,
so we must draw contributing structures to make the point.
Amide Structure
Inclusion of the third resonance contributing structure explains why the amide nitrogen is sp2 hybridized and therefore trigonal planar. Also, the presence of a partial double bond (pi bond) in the resonance hybrid indicates the presence of a restricted bond rotation about the C-N bond. In fact, the measured C-N bond rotation barrier is on the order of 63-84 kJ (15-20 kcal)/mol in amides, enough so that the bond does not rotate appreciably at room temperature. In addition, because the lone pair on nitrogen is delocalized into the pi bond, it is not as available for interacting with Lewis acids such as protons. Thus, amide nitrogens are not basic. In fact, in acid solution, amides are protonated on the carbonyl oxygen atom, not the nitrogen. Finally, the nitrogen lone pair electron delocalization also reduces the electrophilic character (partial positive charge) on the carbonyl carbon atom, reducing amide susceptibility to nucleophilic attack.
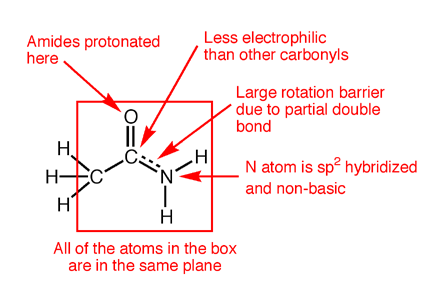
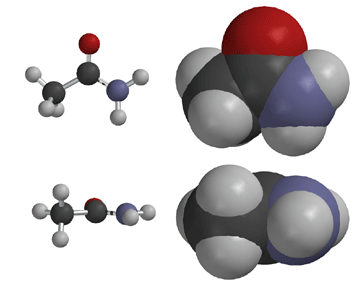
The amide -NH group is a good hydrogen bond donor, while the amide carbonyl is a good hydrogen bond acceptor, allowing primary and secondary amides to form strong hydrogen bonds.
Amide Bonds and Protein Folding
Proteins are made from amino acids connected through mostly secondary amide bonds, which adopt the more stable trans (E) configuration. This is thought to be due to repulsive steric interactions between side chains in the cis (Z) form that are not present in the trans (E) form, along with more favorable dipole-dipole interactions in the trans (E) form. Proline, the only amino acid to contain a secondary amine, produces tertiary amide bonds in proteins, so the cis and trans forms are of similar energy. In fact, proline cis-trans isomerism is now known to contribute substantially to the structure and function of certain proteins.
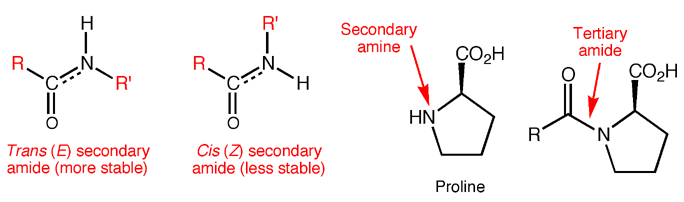
Because amide bonds are resistant to nucleophilic attack, the protein backbone is chemically very stable near physiological pH. Proteins are, however, susceptible to cleavage by certain specially adapted enzymes called proteases that can cleave amide bonds under mild conditions. Some proteases are the therapeutic targets of several recently introduced drugs. Because of the large rotation barrier of the amide C-N bond, one out of every three bonds in a protein backbone does not rotate, significantly reducing the flexibility of the entire chain. In the context of protein folding, this conformational restriction is essential, because it requires much less stabilization energy to stably fold a chain with conformational restriction every third bond compared to a chain with no bond restrictions. The large number of hydrogen bonds possible along a protein backbone provide directional, non-covalent interactions that work in concert with other non-covalent interactions to stabilize a folded structure.
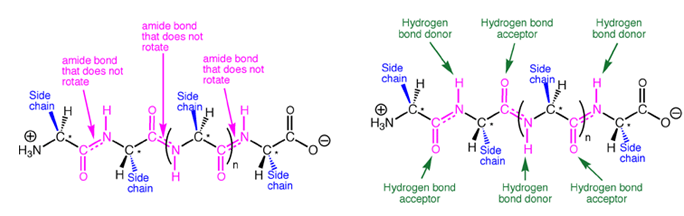
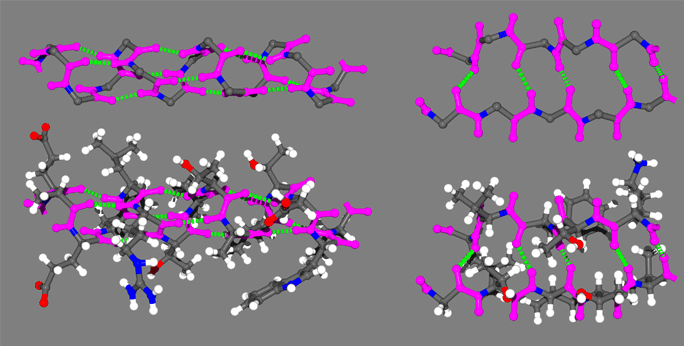
In the above figure are representative protein alpha helix (left) and beta strand (right). The four principle atoms of the backbone amide bonds are highlighted in magenta and backbone hydrogen bonds are shown as broken green lines. The upper structures are the backbone only, with side chain atoms removed for clarity.
A representative complete protein structures in the figure below illustrates just a small sample of the remarkably complex and often quite beautiful folding patterns found in proteins. Here the protein is shown in both a spacefilling and ribbon cartoon model with coils representing alpha helices and the arrows representing strands of a beta sheet. Scientists often use visual aids such as these ribbon cartoons to help them visualize complex structures. Protein structures such as these would not be stable if it were not for the hydrogen bonding and conformational restriction imparted by amide bonds of the protein backbone. It is reasonable to say that the unique structural characteristics of amide bonds have played a relatively large part in shaping the evolution of life on our planet!
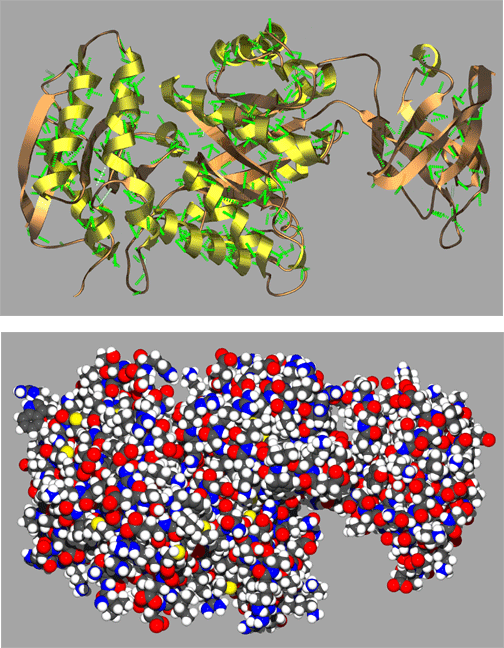